Epikarst is defined as the uppermost zone of dissolution in karst, including whatever soil cover exists. The purpose of this analysis is to explore some of the interactions among geological controls, weathering, biota, moisture flux and soil accumulation in the regolith or critical zone of karst systems.
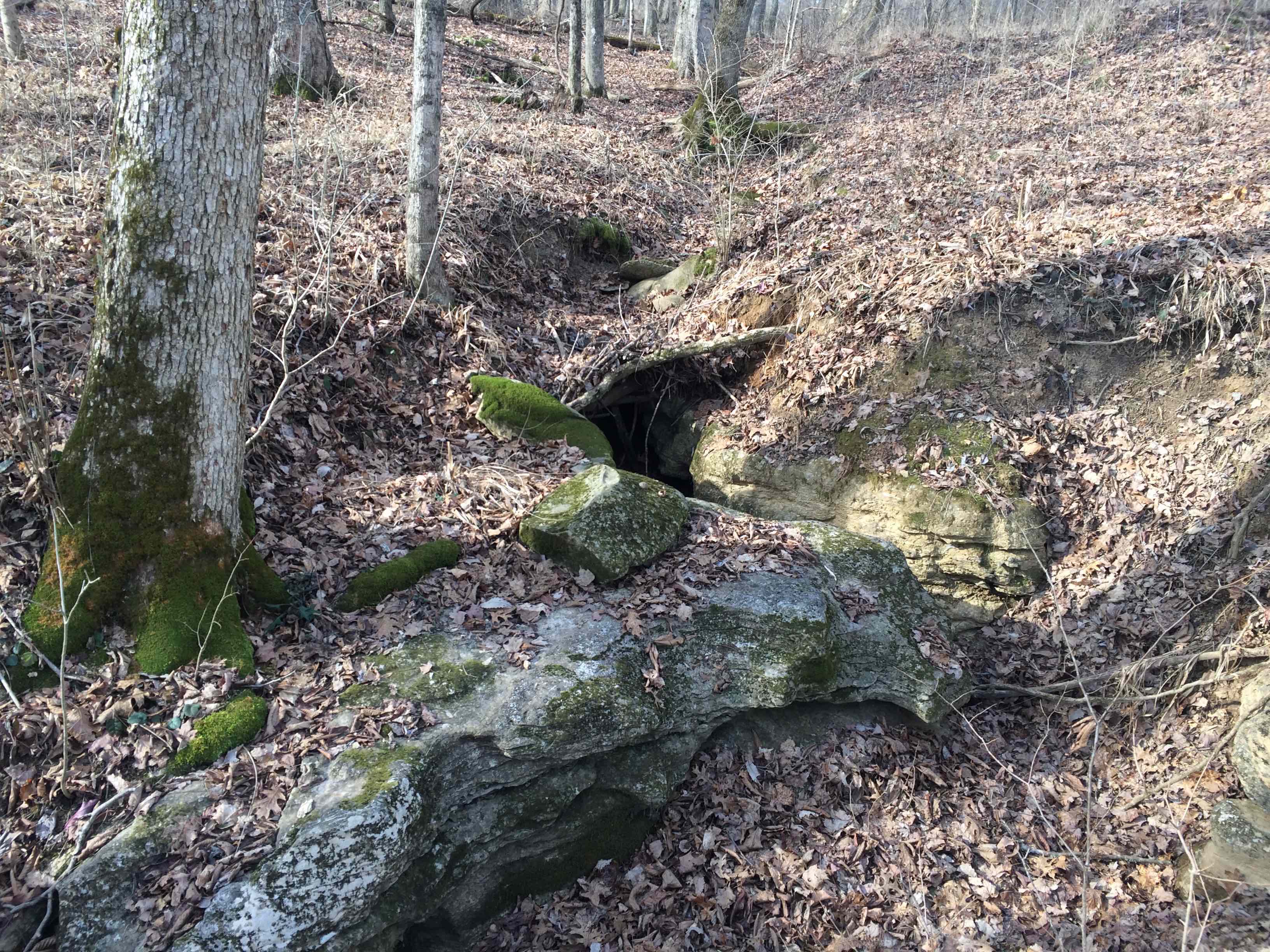
Epikarst exposed by gullying, Bowman's Bend, Kentucky
Figure 1 shows the interactions among geological controls (joints, fractures, bedding planes), weathering, subsurface biological activity, moisture flux, and soil accumulation the earlier stages of soil development in epikarst. The system is dominated by positive feedbacks because in early stages of epikarst development there is limited space for biological activity (e.g., roots), and moisture fluxes are limited by the size of joints, fractures, and incipient conduits. The other positive feedbacks reflect well established relationships among chemical weathering, enlargement of joints, etc., water availability, and organisms. I assume some external (to the system shown) limitations on biological activity and moisture flux.
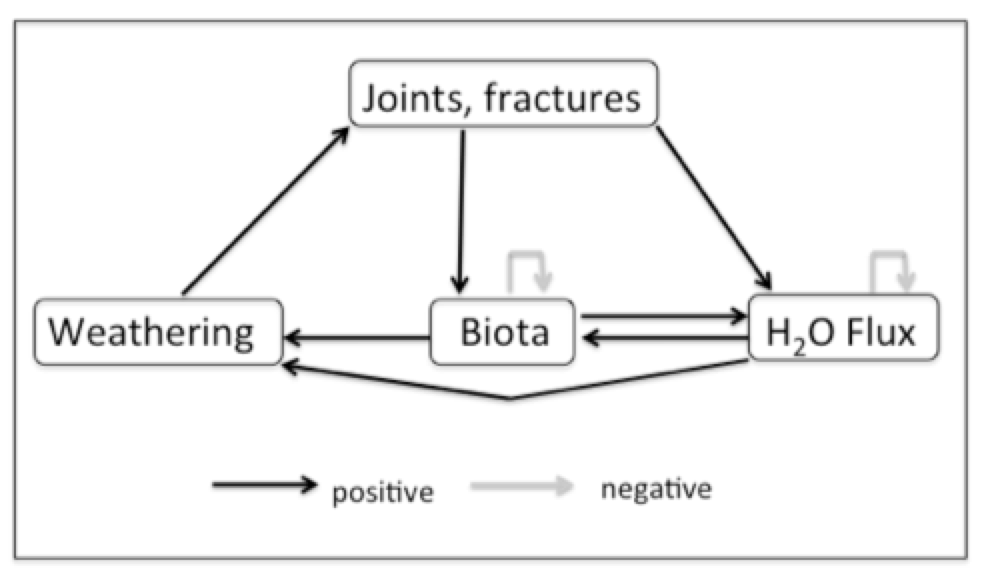
Figure 1. Interactions among structural features (joints and fractures as indicated but also bedding planes and other partings), moisture flow and penetration, biological activity, and weathering (dissolution) in carbonate rocks. These apply in situations where both biota and weathering are moisture-limited, and biota (especially roots) are limited by subsurface accommodation space. Feedback links are summarized in Table 1.
The system shown in Figure 1 is dynamically unstable. Changes such as, e.g., initial establishment of woody plants, will show persistent and disproportionately large alternations in the system state. In the example of roots, their growth accelerates water flux into and within the subsurface (via stem and rootflow, root suction, and promotion of infiltration, which in turn facilitates root growth). Both of these factors enhance weathering, which provides more access for moisture and space for roots. This instability thus promotes continued, and sometimes accelerated, development of epikarst and root zones.
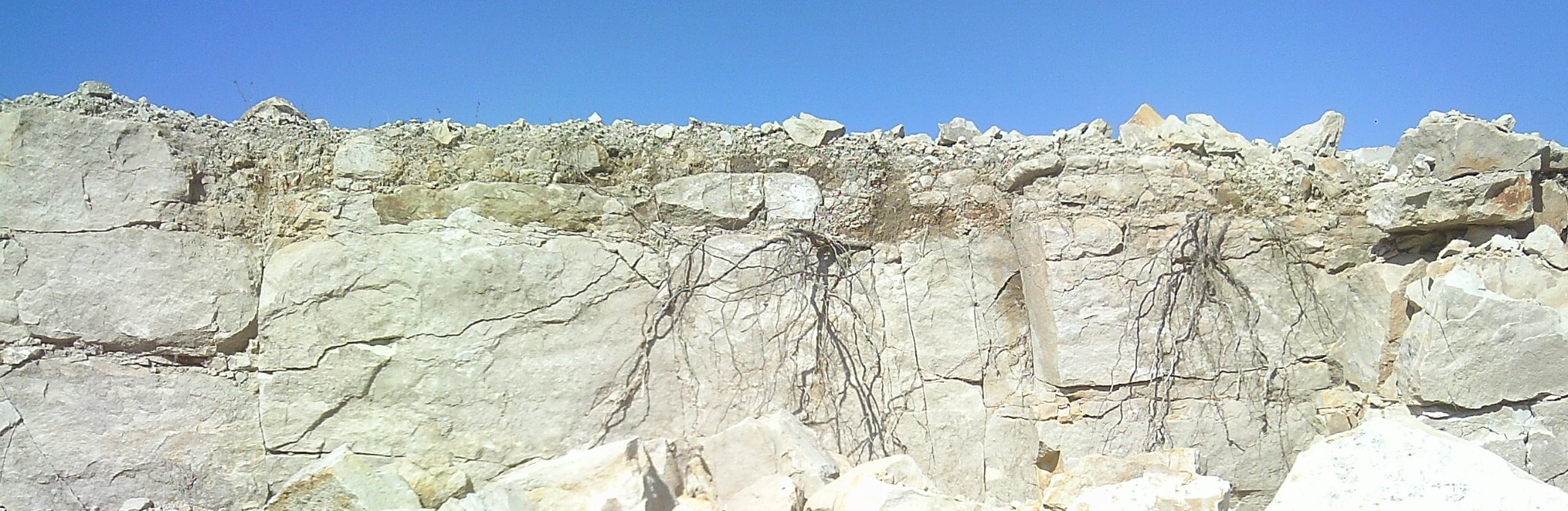
Recently exposed limestone, south central Texas.
The weathering and biological activity also promote development of a soil cover, which is added to the system in Figure 2. Weathering, biotic activity, and subsurface moisture all promote soil development, which in turn improves edaphic conditions for biota and in may cases also promotes weathering, particularly in early stages. Rock weathering may be reduced under thick soil covers, but this is often less relevant in developing epikarst, where the thickest soils occur in widened vertical joints. However, some of the key feedback relationships weaken or become inactive in more advanced stages of epikarst development. In particular, subsurface biotic accommodation space for roots may no longer be a limiting factor, and both weathering and biotic activity may become limited by factors other than moisture supply.
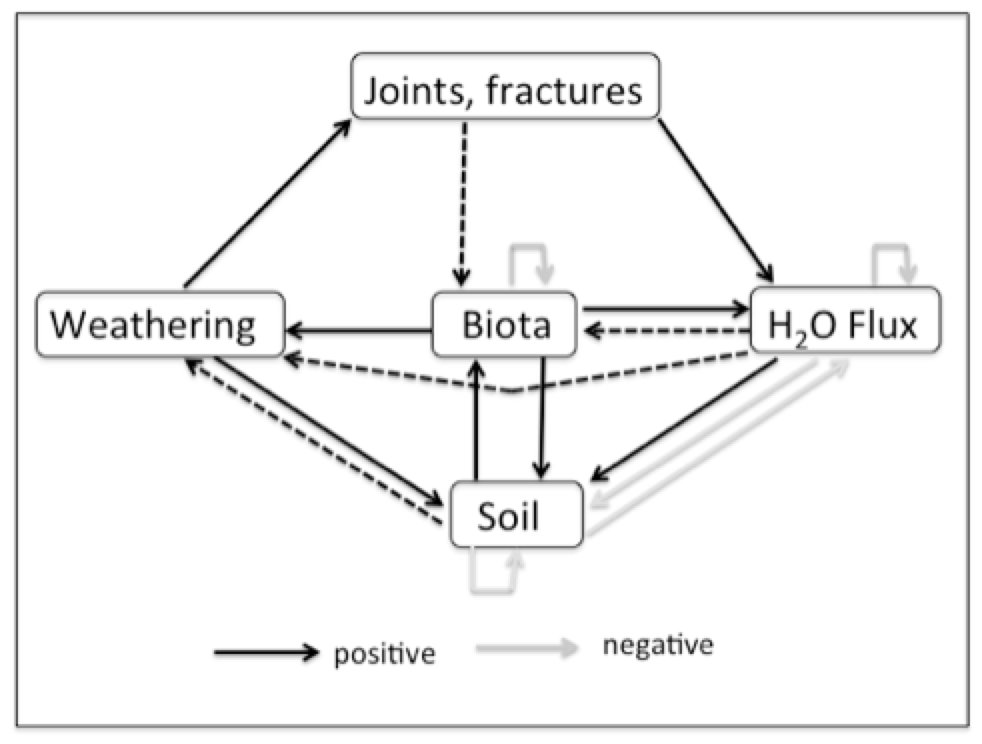
Figure 2. Interaction system with soil added (see Table 1 for explanation). Soil component includes soil development as a surface layer and/or accumulation in joints. Dotted lines indicate links that are positive when present, but that may become inapplicable as epikarst and soil development proceeds.
The system shown in Figure 2 is dynamically unstable when the feedbacks shown as dotted lines are present. However, when they are removed the epikarst system becomes dynamically stable and resilient to small changes or disturbances, implying much more slowly changing conditions.This model suggests that plants (particularly woody plants and trees, which are most likely to have roots in contact with bedrock) are effective ecosystem engineers in epikarst. They help create the dynamically unstable conditions during which belowground biological accommodation space and moisture availability tend to steadily increase, up to the point that subsurface space and water are no longer chronic limiting factors for organisms.
When exposed rock or thin soil epikarst is colonized by plants, ecological filtering and selection presumably favor plants better able to exploit bedrock joints, fractures, and bedding planes. However, the overall enhancement of the epikarst and regolith for biota in general is an emergent outcome of the network of biogeomorphic and ecohydrological interactions.
Table 1. Brief explanation of feedback links in Figures 1-2.
|
Joints, fractures1
|
Weathering
|
Biota
|
Water flux
|
Soil
|
JF
|
|
Effects via H2O & biota
|
Provide space for roots, etc.
|
Enable water penetration & flow
|
|
W
|
Enlarges joints, etc.
|
May be self-limiting in non-carbonate rocks due to depletion of weatherable minerals
|
|
May facilitate in non-carbonate rocks independently of joint enlargement by increasing porosity
|
Enables soil formation
|
B
|
|
Biotic weathering; organic acids; biogenic CO2
|
Competition; resource limits
|
Root channels, evapotranspiration
|
Key factor of soil formation
|
WF
|
|
Promotes weathering unless/until weathering becomes reaction rather than moisture limited
|
Key resource for biota
|
Self-limiting due to climate constraints on H2O supply
|
Water- deposited material (+); Water erosion (-)
|
S
|
|
Promotes weathering up to critical thickness, then inhibits
|
Facilitates biological activitiy via moisture storage, nutrients, etc.
|
Inhibits via moisture storage, reduced open joint volume
|
Possible self-limits due to external constraints (e.g. climate)
|
1Within table, “joints” indicates joints, fractures, bedding planes in general.
Stability analysis--details
The systems shown in Figure 1 and 2 dynamically unstable by the Routh-Hurwitz criteria (see Phillips, 1992; 1999 for explanation in a geomorphology/pedology context and Puccia and Levins, 1985; Cesari, 1971 for a mathematical treatment). However, the system becomes dynamically stable if the dotted-line links shown in Figure 2 are removed. This implies unstable self-reinforcing growth or acceleration of weathering, joint space or size, moisture flux, and biological activity as long as biota are limited (or, looked at another way, stimulated by additional) subsurface accommodation space and moisture supply, and weathering is moisture (rather than reaction) limited. Technically (mathematically) the instability would apply also to reductions in any component, but since weathering and its effects are irreversible, the growth situation is most relevant here.
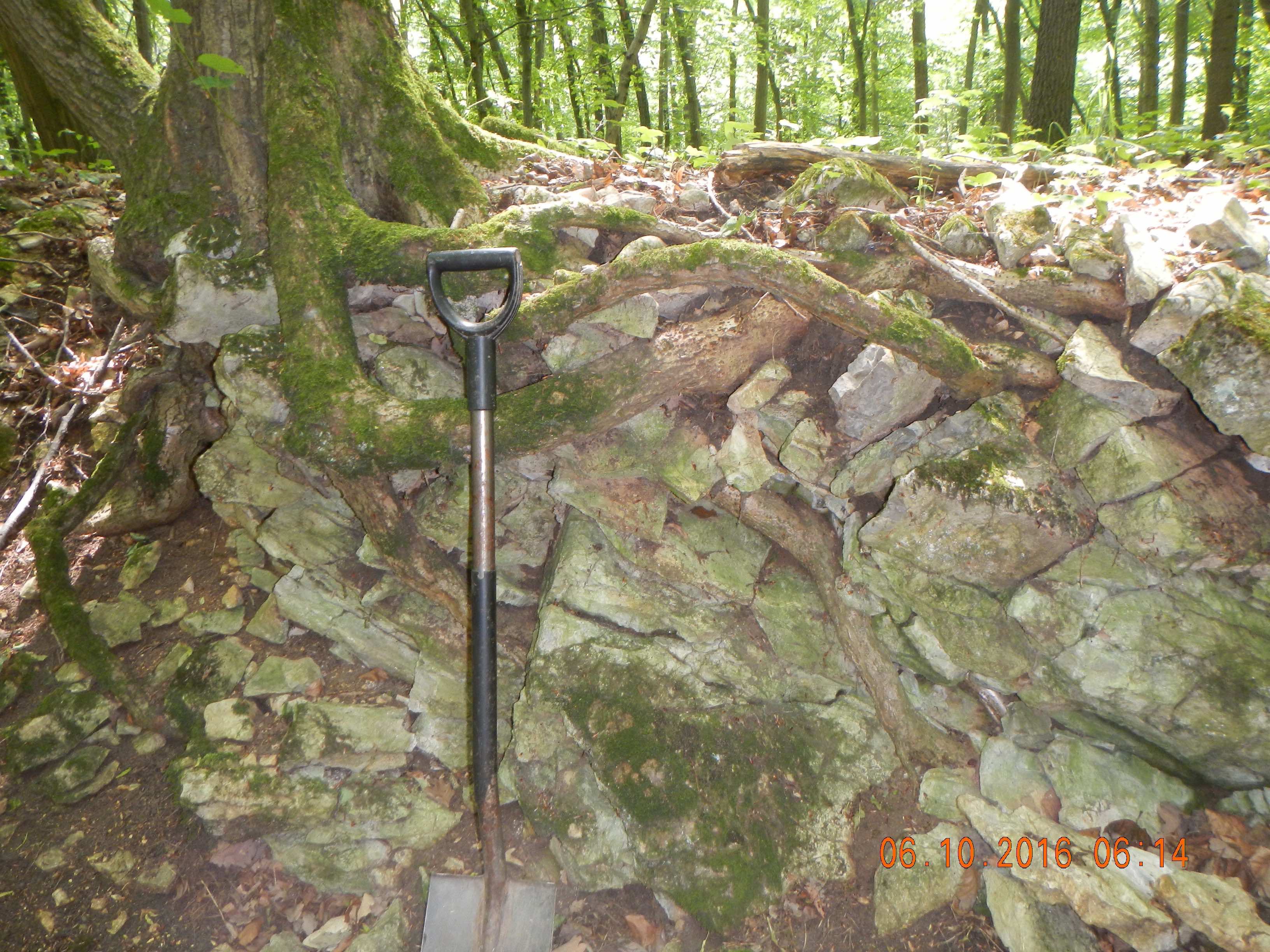
Root-rock interaction, Bohemian karst, Czech Republic.
Including soil does not change the general outcome of the stability analysis, but puts more emphasis on the system reaching a stage where moisture flux, biotic activity and soil development become self limiting (or externally limited). The analysis of this configuration also suggests that plugging of voids with sediment due to water flow tends to enhance the unstable growth phase, while subsurface water erosion promotes dynamical stability.
Table 2 shows the interaction matrix, with links that appear as dotted lines in Figure 2 highlighted. Table 3 shows feedback at level i (Fi) for the without soil (N = 4) and with soil (N = 5) cases. The first Routh-Hurwitz criterion for stability is that all Fi < 0. In Table 4 these equations are interpreted with respect to this criterion.
.Table 2. Interaction matrix for Figures 1-3. Links that appear as dotted lines in Figures 2 and 3 highlighted.
|
Joints, fractures1
|
Weathering
|
Biota
|
Water flux
|
Soil
|
JF
|
0
|
0
|
a13
|
a14
|
0
|
W
|
a21
|
0
|
0
|
0
|
a25
|
B
|
0
|
a32
|
-a33
|
a34
|
a35
|
WF
|
0
|
a42
|
a43
|
-a44
|
+a45
|
S
|
0
|
a52
|
a53
|
-a54
|
-a55
|
Table 3. Feedback at level i (Fi) for the four-component (without soil, Figures 1, 2) and five-component (including soil, Figure 3) model configurations. See Puccia & Levins (1985) for calculation methods. Links that appear as dotted lines in Figure 2 highlighted.
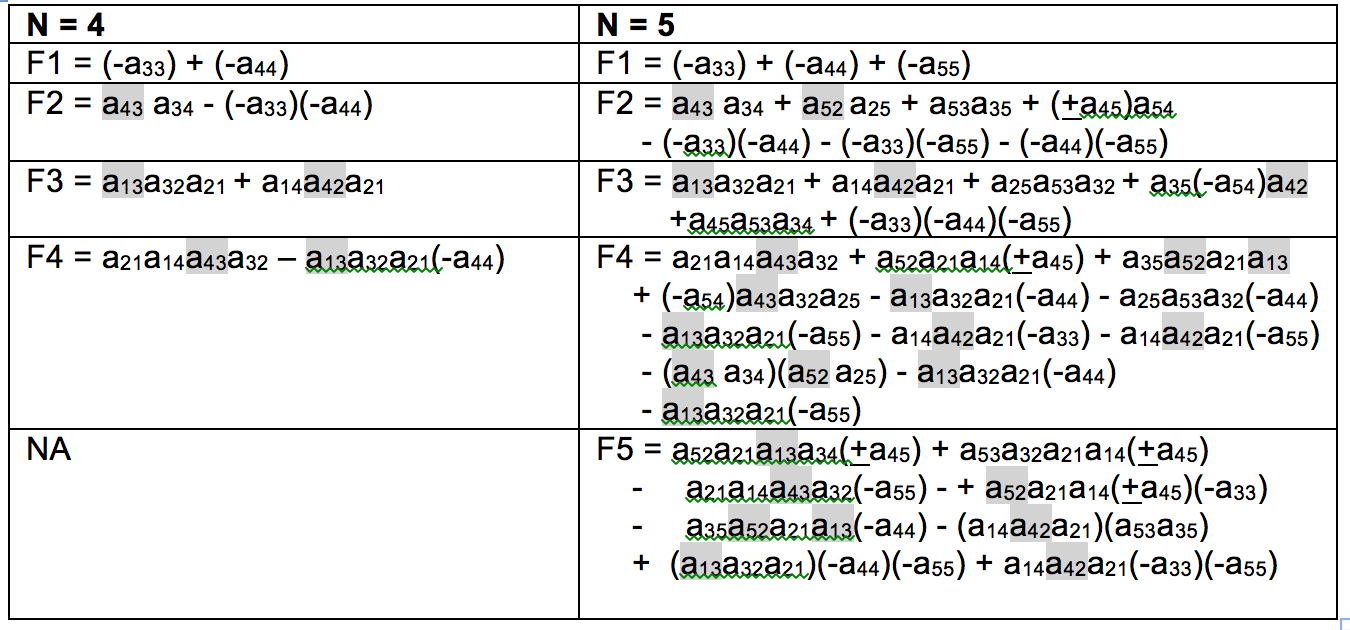
Table 4. Conditions for possible stability (Fi < 0) for Table 3.
N = 4
|
N = 5
|
F1 < 0
|
F1 < 0
|
F2 < 0 if biota not water-limited or if biota, H2O self-limited
|
F2 < 0 if biota, H2O, soil self-limited
F2 < 0 without self-limitations if biota not space-limited, soil effects on weathering negative, soil eroded by water
|
F3 < 0 if biota not space-limited, weathering not H2O limited
|
F3 < 0 if strong self-limitations on biota, H2O, soil and or strong soil inhibition of H2O flux
F3 < 0 if biota not space-limited, weathering not H2O limited; soil-biota-weathering feedbacks not too strong; soil eroded by water
|
F4 < 0 if biota not water or space-limited
|
F4 < 0 if biota not water or space-limited, weathering not H2O or soil-limited, soil eroded by water, soil-biota-weathering feedbacks not too strong
|
NA
|
F5 < 0 if biota not water or space-limited, weathering not H2O or soil-limited, soil eroded by water
|
Key differences relative to non-carbonate systems
Many of the feedbacks and interactions described above could be relevant to any, or many, critical-zone or regolith situations. However, some key potential differences associated with non-karst, non-carbonate settings are shown below (Table 5). The models above are based on an assumption of carbonate rock with only minor amounts of insoluble material, and of sufficient thickness to accommodate full development of the root zone.
Table 5. Potential key differences, carbonate vs. non-carbonate settings.
Difference (non-carbonate relative to carbonate rock)
|
Model implications
|
Weathering may be self-limiting due to depletion of weatherable minerals.
|
New link (-a22) could increase likelihood of stability
|
Moisture flow & penetration may be facilitated by weathering independently of fissure enlargement due to increased porosity
|
New link added
(a24) could decrease likelihood of stability
|
Soil clogging of, or erosion from, fissures, conduits less important (may depend on macropores)?
|
Sign change: a54 = 0?
|
Key questions
•The concept of moisture-limited vs. reaction-limited weathering is well establishing in the literature on development and enlargement of karst fissures and conduits. There is o reason to expect that the phenomenon does not occur in epikarst development as well--is there?
•Does vegetation indeed become less water limited as epikarst evolves?
•What is the relationship between soil thickness and weathering rate in epikarst? Does the "humped" soil production function apply? Exposed limestone weathers quite readily without any soil sover, and the irregular, discontinuous geometry of the weathering from and concentration of soil in enlarged joints may obviate or obscure any relationship between soil thickness and rock weathering rates, as water and biota are rarely far removed from rock.
•What is the role of subsurface moisture flow in depositing vs. eroding soil and sediment?
Finally, the key question is whether there exists detectable threshold or mode switch from unstable rapid growth of epikarst driven by ecosystem engineer effects of tree roots, to stable steady-state or slow growth.
The bottom line
I am confident that the interactions among rock weathering, moisture flux, biotic activity (especially roots), and soil/regolith development operate such that plants function as ecosystem engineers (EE) to produce conditions increasingly suitable for woody vegetation, up until the point that factors other than moisture and rooting space become limiting. I am also confident in the fundamental dynamical instabilities that underlie the phenomenon.
As the questions above indicate, I am less confident that I have all the details correct, and how and whether the stable condition occurs. I am moderately confident that the ecosystem engineering is non-specific--that is, the process creates generally better conditions for any woody plants and vegetation in general suited to the habitat, rather than the engineer species specifically. To the extent that they are self-replacing this is likely due to the availability of propagules rather than the EE effects. That is if, say, a chinquapin oak facilitates weathering and soil formation and improves plant habitat (and here in central Kentucky, they do) and another of the same tree replaces it, this is because there are plenty of chinquapin acorns around, not because the soil and epikarst has been made chinquapin-friendly relative to other species.
The biogeomorphic EE discussed here is contingent and emergent: Contingent on an epikarst environment (I have written on contingent biogeomorphic EE in karst here). Emergent in the sense that it derives from the particular biogeomorphic interactions in epikarst rather than being inherent in the extended phenotype of the EE organisms.
References
Cesari, L., 1971, Asymptotic Behavior and Stability Problems in Ordinary Differential Equations: New York, Springer-Verlag, 271 p.
Phillips, J.D. 1992. Qualitative chaos in geomorphic systems, with an example from wetland response to sea level rise. Journal of Geology 100: 365-374.
Phillips, J.D. 1999. Earth Surface Systems. Complexity, Order, and Scale. Oxford, UK: Basil Blackwell.
Puccia, C.J., Levins, R., 1985. Qualitative Modeling of Complex Systems. Harvard University Press, Cambridge, MA.
(posted 22 September 2017)