In many of my writings I advocate an alternative to reductionist approaches to science. By alternative, I mean a complementary, different way of doing things, not a replacement for reductionism. Many excellent reviews of scientific approaches, viewpoints, and methodological stances exist by historians, philosophers and sociologists of science, and by scientists themselves. I do not intend to review or critique these various approaches here. Further, I have no intent to deny the value or necessity of reductionist science. The crux of my argument is that a reductionist approach, by itself, is inadequate or incomplete for understanding Earth.
The American Heritage Dictionary defines reductionism as an attempt or tendency to explain a complex set of facts, entities, phenomena, or structures by another, simpler set, and provides a quote from John Holland:
For the last 400 years science has advanced by reductionism ... The idea is that you could understand the world, all of nature, by examining smaller and smaller pieces of it. When assembled, the small pieces would explain the whole.
We can distinguish between the practice of reductionist science, pursuing understanding and meaning using the atomistic tools of reductionism, versus a stronger philosophical/theoretical stance. The latter holds that complex systems or ideas can always be reduced to a set of simpler, more fundamental components, and that fundamental or first principles always reside at the smallest level. A belief that reductionism is the primary and preferred path to scientific explanation is by no means rare among scientists, but is less prevalent than a simple acceptance of reductionism as a valid methodology or tool. For instance, the population of biologists using reductionist methods is much larger than the population of biologists who believe that all biological phenomena can ultimately be explained by mechanisms operating at the molecular (or smaller) level.
I am arguing, then, not against reductionism as a valid and useful methodology. In fact, I would argue that it is, and always will be, necessary. I do contend that reductionism by itself can never fully explain Earth surface systems, that reductionism is often not be best approach for some problems, and that where alternatives exists, reductionist methods and interpretation are not necessarily superior or preferable. Why not?
Multiple Scale Causality
In the experimental and laboratory sciences, observed patterns and processes can sometimes be conceptualized straightforwardly as macroscale manifestations of microscale processes. In field-based sciences, however, it is more typical that observed patterns or system states are attributable to multiple processes and controls. Further, those multiple controls operate at multiple spatial and temporal scales--both larger and smaller than the scale of observation.
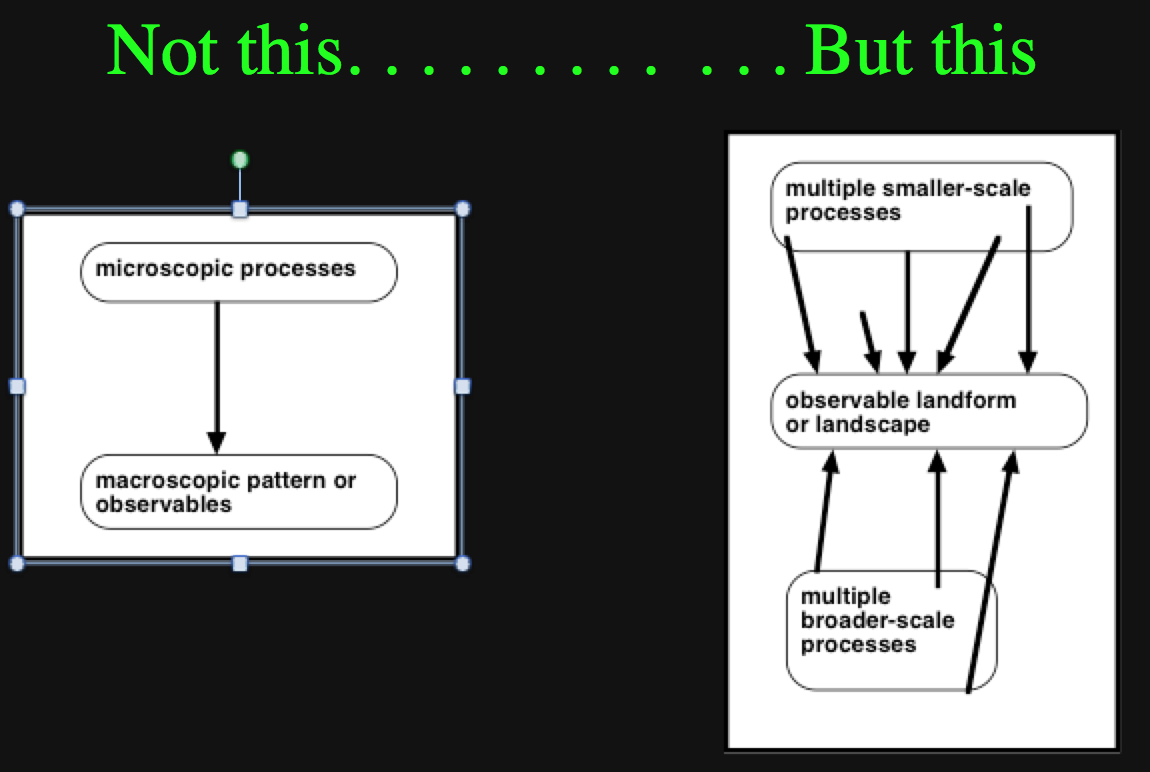
Landforms are a good example. They are created by a number of processes acting at molecular and granular scales (e.g., biological and chemical weathering, erosion, and sediment transport), by factors operating over much broader scales (climate, tectonics), and by controls such as geological structure, which may operate at scales ranging from granular to continental. This notion of multiple scale causality (MSC) not only recognizes multiple processes and controls acting at a range of scales, but also recognizes (in contrast to a strict reductionist approach) that the relevant ‘‘first principles’’ may operate at levels other than the smallest microscales.
Even among Earth and environmental scientists acknowledgement of MSC is not universal, and some emphasize causality at single, particular scales. However, such emphasis does not necessarily imply the rejection of causality at other levels. The evolutionary ecologist, for instance, does not necessarily deny the importance of microscale processes even as she focuses on macroscale evolutionary phenomena. Similarly, the dynamic climatologist, concerned chiefly with atmospheric physics, is unlikely to reject the relevance of synoptic climatology. Recognition of MSC is often implicit: though particular causal agents and scales may be emphasized, causality at other scales is at least indirectly acknowledged. Accordingly, despite the tradition of reductionism, in the field-based sciences claims of causality residing entirely at a given scale are rare.
Ideally, we directly confront MSC, by deliberate attempts to minimize effects at some scales and maximize effects of others, or by isolating scale-contingent causes and explanations. Even when MSC is explicitly recognized, we often seek causal explanation or representation (cartographic, mathematical, conceptual, epistemological, or otherwise) that can accommodate the vast range of potentially relevant scales. Such representations can be reductionist, as in attempts to understand climate on the basis of the fundamental equations of motion or landscape evolution in terms of microscale process mechanics. But reductionists have not cornered the market for attempts at seamless cross-scale representations. Certain complex systems theories have been proposed as covering laws or metaprinciples for geomorphology, biogeography, biodiversity, and evolution; or even nature in general.
On the other hand, some (including myself) maintain that fundamental changes in controls over process-response relationships occur as spatial and temporal scales change, and that unitary, seamless explanation across scales is not only difficult, but fundamentally impossible or unfeasible.
One approach to MSC is via study design. Some are specifically geared toward minimizing—ideally, eliminating—the influence of all but one or a handful of controls or variables. In other cases, investigators simply narrow the focus to minimize variability in broader-scale factors or expand the focus in the sometimes realistic hope that local-scale variations will cancel each other out or be obscured by broader scale factors. Another strategy for coping with MSC involves analytical methods to disentangle the explanatory contributions of variables operating at a range of scales, or at least isolate the variance contribution of a particular scale of interest.
Eco- and geoscientists sometimes cope with MSC, especially in practical applications, by acknowledging that variability is influenced by multiple sources and is manifested at a variety of scales, and then seeking to identify the dominant or critical scales in the context of a particular problem. Geostatistical analysis, for example, is often aimed at identifying the area or distance over which spatial variables are dependent or independent. Other approaches seek to determine the resolution or scale at which variance of the phenomenon of interest is minimized—e.g., determination of representative elementary areas and volumes in hydrology.
An explicit approach to MSC that has been applied in ecology, biogeography, geomorphology, and other fields is hierarchy theory. The fundamental idea is that a hierarchy of spatial scales is identified. In this hierarchy, at any given level of i, there exist controls or influences operating at adjacent larger and smaller scales, i -1, and i + 1, which may be observed at, and are relevant to, level i. Processes at levels further removed, at either broader or finer scales, operate either too rapidly and finely, or too slowly and broadly, to be observed or manifested at i. Ideally, these hierarchies allow functional relationships to be transferred up and down the scale hierarchy.
Other, analytically explicit, efforts to engage MSC, include local forms of spatial analysis and entropy decomposition. These generally deal with the issue of assessing the contribution of global and local factors (defined broadly) to the structure and/or variability of spatial patterns.
Independently of formalisms such as hierarchy theory and MSC, defining geographical regions—ecoregions, biomes, agricultural regions, physiographic provinces, etc.--is based on attempts to separate groups of local-scale (within regions) and broader-scale (between regions) controls. In other words, regional delineations attempt to minimize within-region (and maximize between-region) variations.
Fundamentally, however, the basic point is this: Real-world Earth surface systems are characterized by multiple scale causality, reductionist approaches alone are not sufficient for fully understanding them. The other side of the coin is that methods that deal with the most atomistic scales—reductionist methods—are necessary for understanding them.
All Other Things Are Never Equal
Basic two-way, cause-and-effect relationships in the sciences are generally based on the principle of ceteris peribus—all other things being equal (AOTBE). For example, AOTBE, increasing CO2 concentrations in the atmosphere have a fertilization effect, increasing plant productivity. Of course, plants ability to use the additional CO2 depends on their having adequate supplies of sunlight, water, and nutrients. As greater atmospheric CO2 influences climatic variables that in turn influence plant growth, and the activities that change atmospheric chemistry also modify nutrients and other edaphic factors, all other things are not equal. In ESS, all other things are never equal. Why? Because everything is connected to everything else. This maxim has been cited as the First Law of Ecology, the First Law of Environmental science, and the First Law of Geography.
Everything is not literally related to everything else in the sense of direct, tracable, causal, significant links between any two objects, processes, systems, or places. But everything is related to everything else in the sense that Earth system are characterized by multiple, interrelated components and controls. Our world is seen and analyzed by geoscientists and ecoscientists in terms of maps, webs, matrices, flow charts, multiple equation systems, and other representations of multiple, interconnected, mutually adjusting objects or phenomena. Places and environmental systems are understood as the outcome of multiple, interrelated forcings and controls and complex histories.
All humans (or other species), for instance, share common genetic ancestors, and all of us play our roles in the carbon/oxygen cycle with every breath we take, alongside other processes and entities, animate and inanimate. Additionally, long-range interrelationships and teleconnections are typical of ESS: Sea surface temperature anomalies in the equatorial Pacific or the North Atlantic have climatic and oceanographic repercussions around the globe. Commodity price changes in China or Chicago affect land use, soil erosion, and nutrient budgets on fields and paddocks around the world. Coastal landforms in British Columbia or Sumatra may be linked to long-ago, far-away, undersea earthquakes or landslides that triggered tsunamis.
Bailey, Bones & Butterflies
More than a decade ago, writing along these lines, I spoke of the vast web of connections using the metaphors of Bailey effects, bones, and butterfly effects. The “George Bailey Effect” is named after the protagonist of Frank Capra’s 1946 film ‘‘It’s a Wonderful Life,’’ based on a story by Philip Van Doren Stern. George Bailey suffers a run of terrible luck and self-doubt. In despair and attempting suicide in the belief that his life has not been productive or worthwhile, Bailey is rescued by a guardian angel. He is shown an alternative reality; what his community would be like if he had never been born—in the story, a much more desperate, depraved, and depressing place, because the good but relatively routine acts of kindness, bravery and common sense George Bailey committed had not happened. The message: apparently minor actions of an individual may have ripple effects and chain reactions that produce dramatically different outcomes.
Bailey Effects are based on conditionality. Whether or not George saves his brother Harry from falling through the ice as a child, determines whether a loaded troop ship is, or is not, saved by the heroic actions of Harry Bailey later on. The conditionality type of contingent connectedness is common in nature. For example, if the surface cold air layer in a winter inversion is shallow rather than deep, then the precipitation is freezing rain instead of sleet. The freezing rain may in turn result in an ice storm, the effects of which then influence forest species composition (Lafon et al. 1999). Many ESS are conditional with respect to the occurrence, frequency, and timing of factors such as storms, fire, sea surface temperature anomalies, or inherited effects of landform singularities.
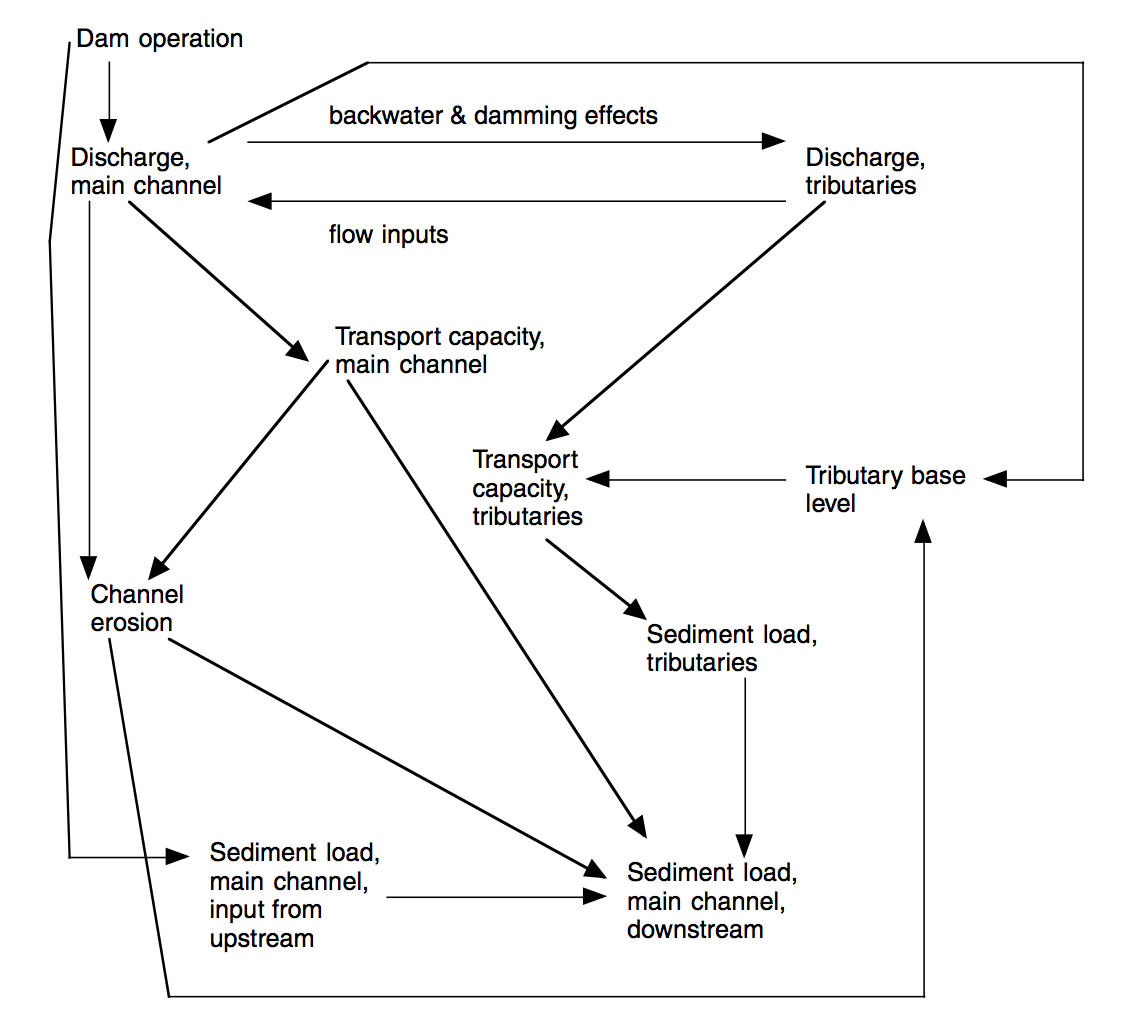
Complex interrelationships between key geomorphological factors downstream of a dam (Figure 2 from Phillips, J.D. 2003. Toledo Bend Reservoir and geomorphic response in the lower Sabine River. River Research and Applications 19: 137-159).
The ‘‘bones’’ connections indicate physical, mechanistic, causal interconnectedness, based on the metaphor of a skeleton: the foot bone’s connected to the ankle bone, the ankle bone’s connected to the leg bone, etc. “Bones” connectivity may be long and complicated, but the chain of interrelationships is clear. This type of relatedness is also common in ESS, and arises mainly due to the presence of numerous components or degrees of freedom, hooked together in long, interconnected chains of causality. Visual evidence is apparent in any detailed diagram of the carbon or nitrogen cycle, a watershed system, an ecosytem, marine food web, etc.
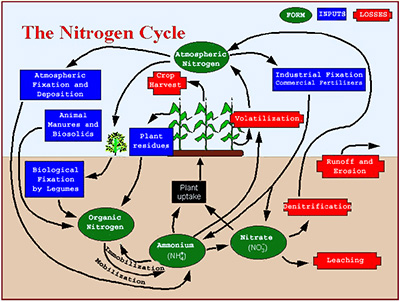
Nitrogen cycle in soils--simplified! (http://www.extension.umn.edu).
“Butterflies” refers to the famous butterfly effect metaphor from chaos theory. A presentation at a scientific meeting by Edward Lorenz in 1972 was titled ‘‘Does the flap of a butterfly’s wings in Brazil set off a tornado in Texas?’’ This was based on Lorenz’s now-famous early work on chaotic dynamics in the atmosphere, where he showed the fundamental equations of motion to be highly sensitive to initial conditions. This sensitivity and chaotic divergence over time gave rise to the notion that a tiny change at a given place and time—e.g., the flap of a butterfly’s wing—might lead to disproportionately large results, far away. Thus ‘‘the butterfly effect,’’ one of the favorite metaphors of chaos and complexity theory. As an aside, as far as I know, no one has ever followed up on my suggestion that “an interesting exercise in trivial geography and chaos theory would be to catalog citations of the butterfly effect, with a map of the different locations of the butterfly and the storm.”
Many environmental systems are, or can be, deterministically chaotic. This means that the effects of minor variations in initial conditions, or of small perturbations, are exponentially magnified over time. Butterfly effects imply elaborations of both Bailey Effects and ‘‘bones’’ connections. Chaos (= dynamical instability) produces the possibility of Bailey effects disproportionately large and long-lived relative to the initial stimulus, and causal webs more subtle and complex than leg bones connected to knee bones connected to thigh bones suggests. Butterfly effects also make possible historical connectedness via ‘‘memory,’’ in the sense that the effects of variations or disturbances that are no longer detectable are manifest in the current state of the system.