Some recent kayak trips on the North River near Beaufort, NC (which, naturally enough, is north of North River, SC, but strangely enough well south of the other North River, NC, and even more strangely, south of the South River in the same county) revived some nagging questions about the source of sediment to coastal marshes.
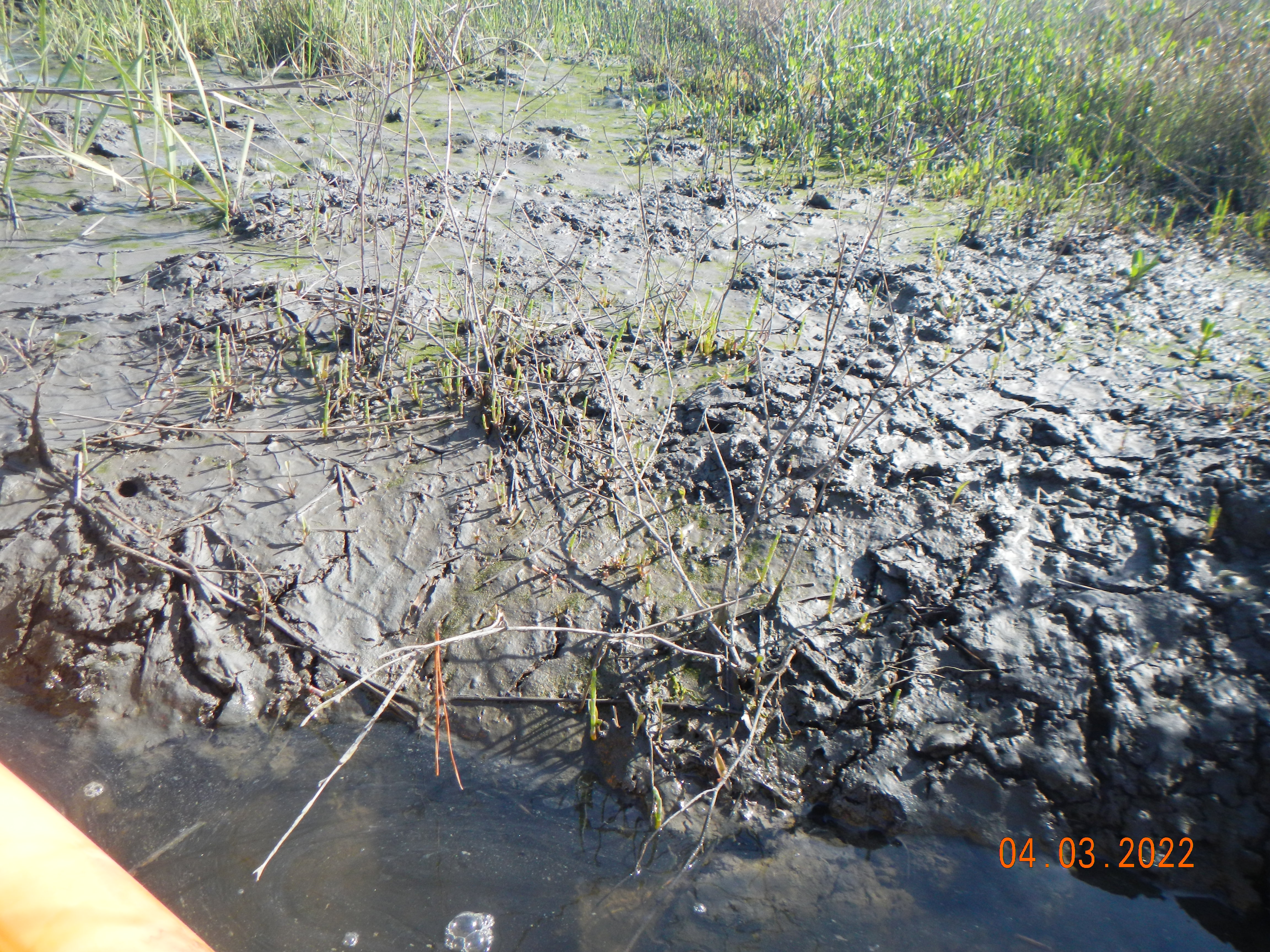
Freshly deposited mud on the North River marshes.
Most of my work in this context has dealt with larger rivers on the Atlantic and Gulf Coastal Plains (drainage basins >15,000 km2) addressing (among many other things) how much fluvial sediment is delivered to estuaries and coastal wetlands, and where within those drainage basins it comes from? Some updates and reminiscences were covered in this post. Essentially, my work (and many others) has found that in many river systems much of the sometimes-considerable sediment loads from the upper watersheds never reaches the coastal zone, being stored as alluvium in lower river reaches. Much of what does reach the coast derives from coastal plain sources near the coast, not from upriver.
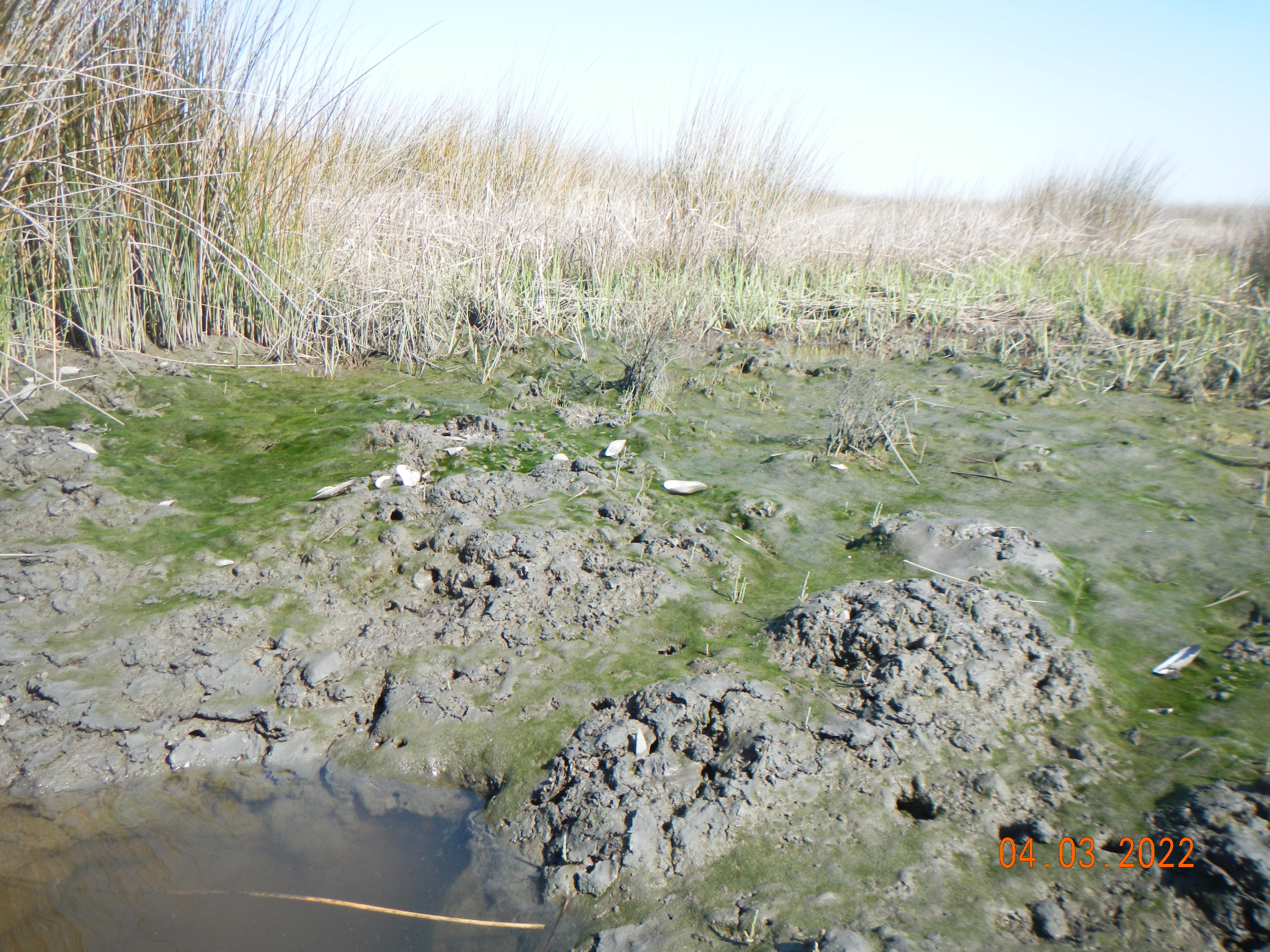
Algal biofilm (and mussel shells from river otter meals) is visible at this spot where ebb tide flows have high enough velocity to inhibit deposition.
But coastal wetlands, some extensive, occur in areas where there is very little upland runoff and sediment contributing area at all. At least some of these are not only getting sediment deposits, but also getting enough to keep pace with rising sea-level. Where is this marsh mud coming from? Let’s take a look at that, using North River as an example.
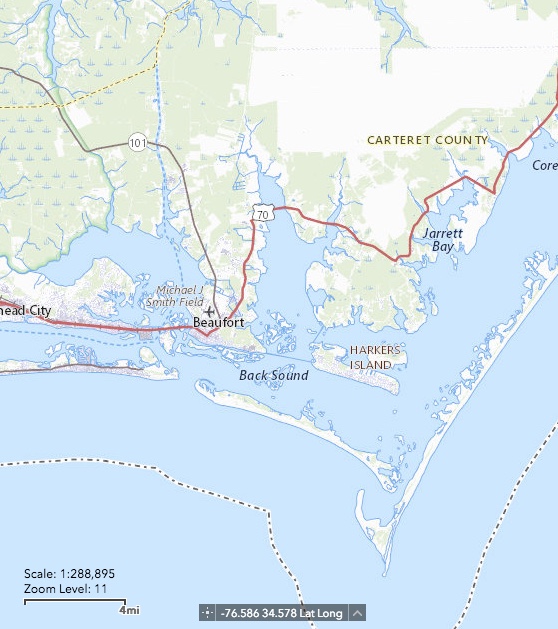
North River (center of map), as shown on U.S. Geological Survey’s National Map.
North River is a small estuary in Carteret County, tidal along its entire length (<15 km to Core Sound). The drainage area is hard to ascertain, because a number of drainage ditches and canals obscure the natural drainage patterns and reroute flow paths. However, the freshwater drainage area is minimal, and astronomical tides are the main source of water flux in the marshes. In the upper North River (upstream of the U.S. highway 70 bridge) the marshes are dominated by black needlerush (Juncus roemerianus). Saltmarsh cordgrass (Spartina alterniflora) occurs along some bank/shoreline fringes and low spots. A handful of other regionally typical salt-tolerant species occur. Groundsel bush (Baccharis halimifolia), which has long been present, appears to have recently expanded and seems to continuing expansion, dominating some patches and snuggling in with Juncus along many banks.
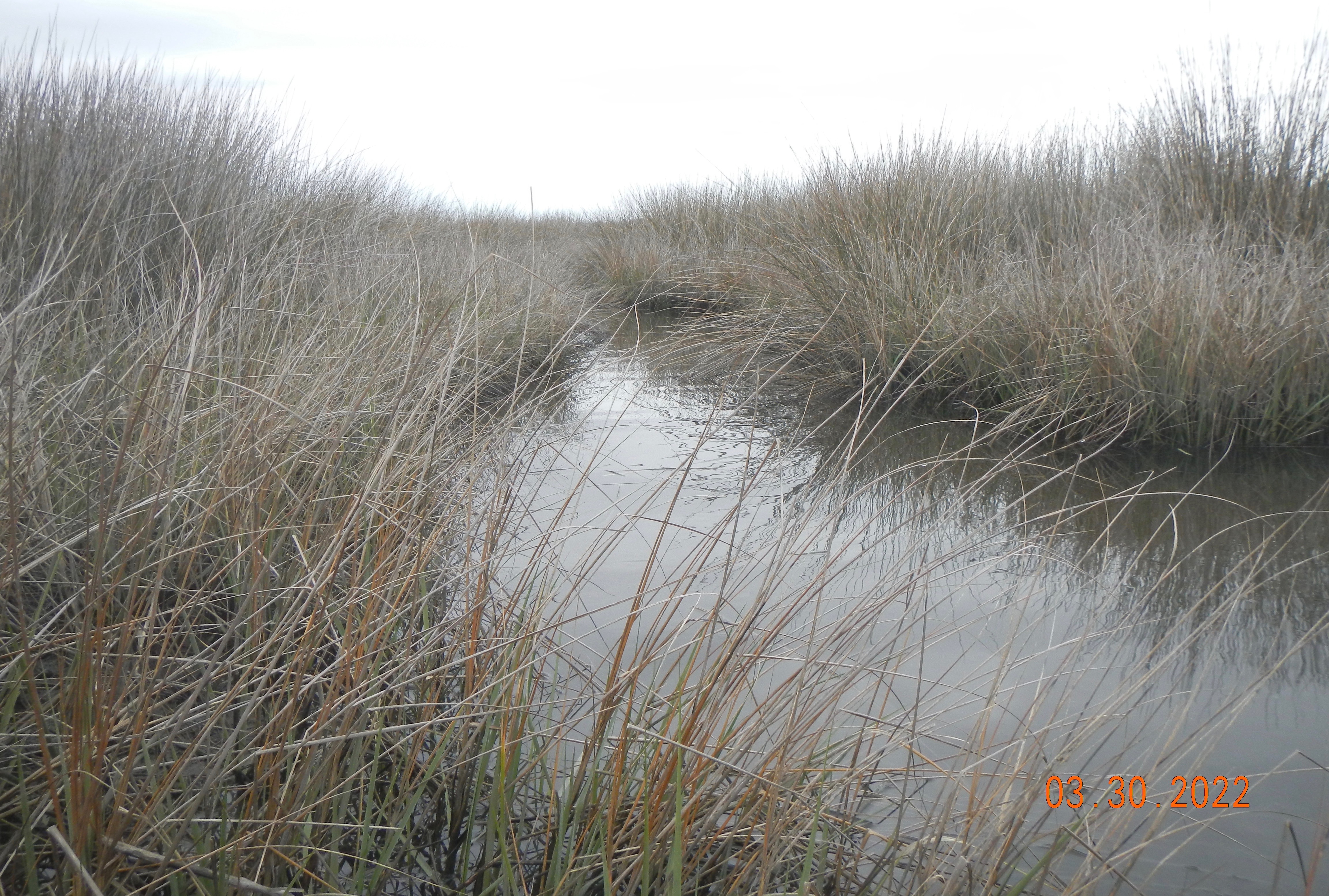
Juncus roemerianus marsh along the North River.
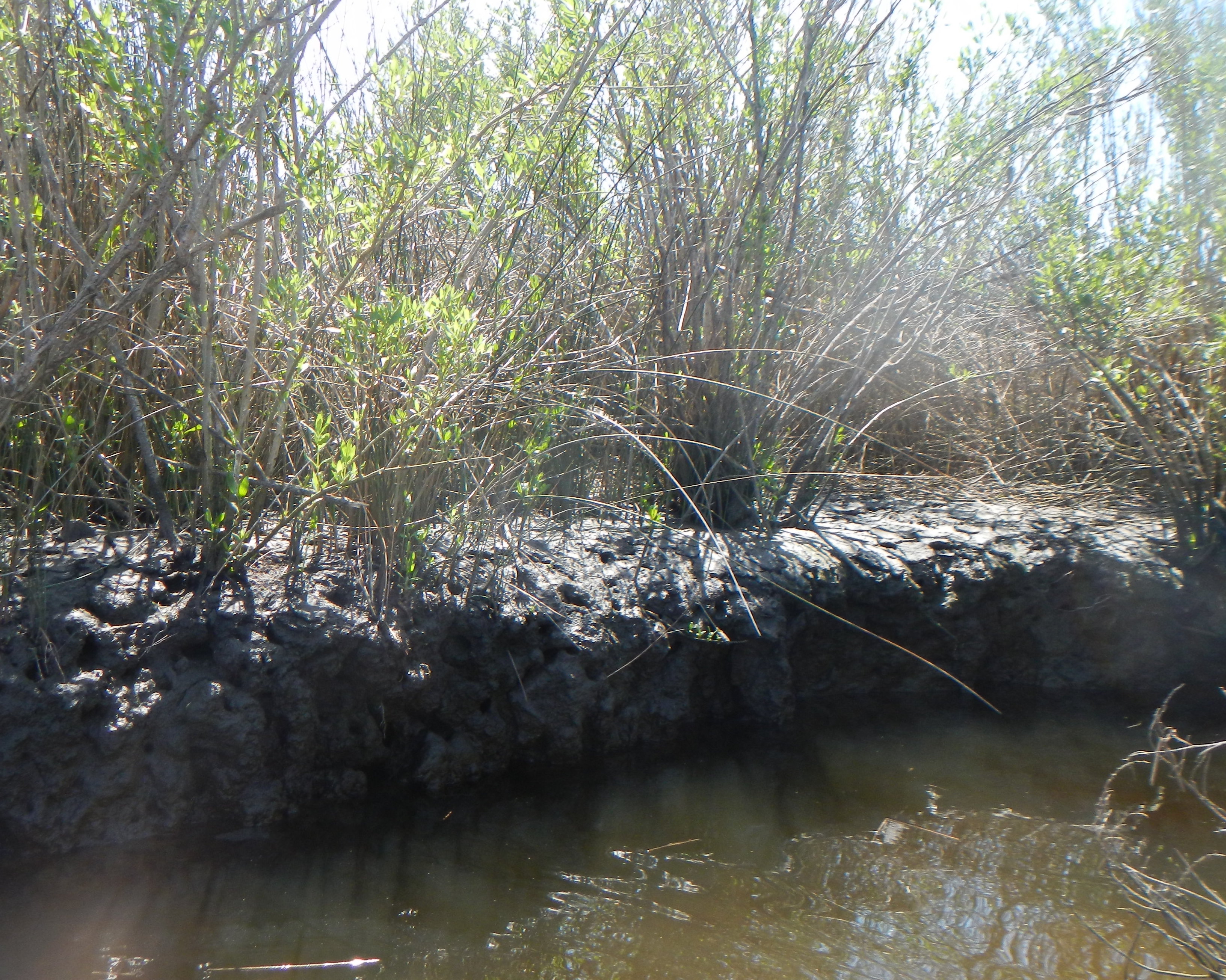
Mud deposits and scarped bank.
Potential sediment sources
First, we’ll identify all conceivable sediment sources, and evaluate them one-by-one.
1. Fluvial delivery from inner coastal plain or piedmont? Nope. North River has a limited drainage area, entirely within the outer coastal plain, and no connection to larger rivers.
2. Fluvial delivery from local sources. Possible. Portions of the watershed are developed for agriculture, forestry, and residential use. In the upper river (upstream of Felton Creek) the only potential source is Open Grounds Farm, a large corporate farm established in 1974, with an area of nearly 18,000 ha of mainly row crops. The farm is entirely artificially drained, and the drainage ditches connect to a constructed channel that bypasses the upper North River, though some connection during wet conditions is possible. Most of the farm is mapped as the Deloss soil series, which ranges from sandy clay loam to loamy sand in texture, which seems an unlikely dominant source for the mucky silty clay comprising the most recent (spring 2022) surface deposits. The soil in the marshes is mapped as the Hobucken series, which has muck and mucky fine sandy loam surface horizons, overlying fine sandy loam, but in the field it is difficult to spot any sign of sand.
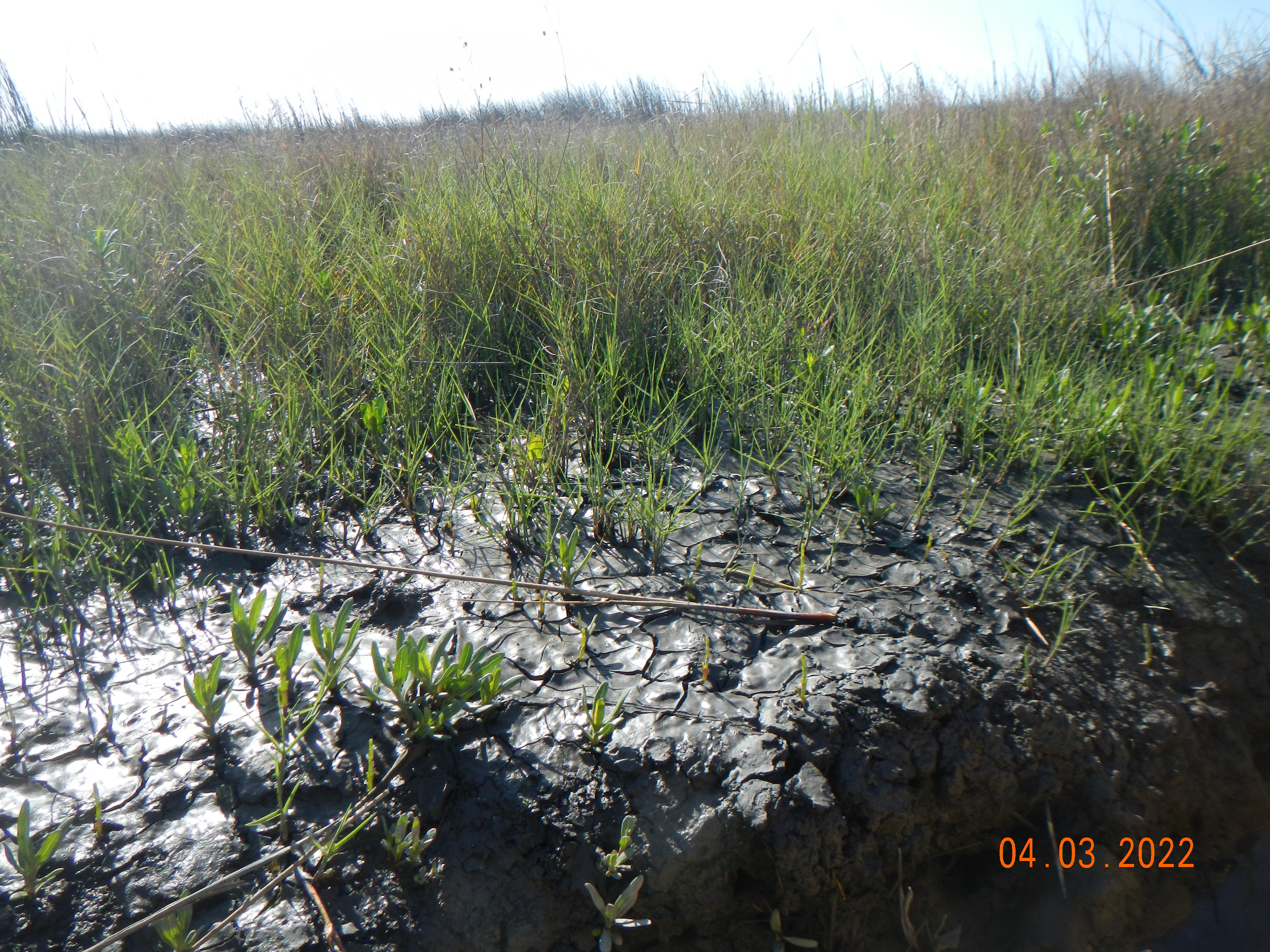
Not much sand here!
I don’t think this is a major source, but I can’t rule it out. First, a lot of my work from back in the day shows that more erosion is occurring on the coastal plain than has traditionally been thought, and that even in larger Piedmont-draining rivers, coastal plain sources are the dominant supply of inorganic sediment in the lower river reaches (see my River Sediment Delivery to the Coast post from December, 2020). Also, in the next-door Newport River estuary, Mattheus et al. (2009) showed that the direct connectivity from tree farming in the upper watershed to the river facilitated a rapid response to erosion and sediment inputs.
Several case studies, especially from the Chesapeake Bay region, show marshes growing in response to accelerated erosion and sediment production from 18th and 19th century land-clearing, in many cases within small coastal watersheds without much interior drainage area (e.g., Kirwan et al., 2011).
3. Estuarine and marine sediments. As a tidal system, also strongly influenced by wind, landward or inland transport of estuarine sediments occurs, and also probably offshore sediment as well, as the mouth of the river is close to Beaufort Inlet.
4. Aeolian input. Inputs of wind-transported sediment are not usually considered significant, except in marshes on the back side of barrier islands or otherwise in proximity to sand dunes. However, studies of dust inputs to soils in many locations have typically found significant aeolian contributions even where wind erosion and aeolian processes are not considered significant. Dust is also the major source of bioavailable iron to the ocean. Charles Darwin (1846) published a paper on dust falling on vessels in the Atlantic Ocean. There is no reason to suspect that the North River and its wetlands do not also get some dust input. Further, studies on the N.C. coastal plain have found significant aeolian erosion from croplands (Pease et al., 2002; Gares et al., 2006). Thus wind-blown sediment from Open Grounds and several other nearby corporate farms is possible, as well as dust from father afield.
5. Marsh bank erosion. The area includes numerous scarped marsh edges and tidal creek banks, and slumps from recently eroded material are evident in many locations. This source is essentially a recycling of sediment previously deposited on the marsh.
No measurements of bank erosion in the North River have been made, but field evidence is abundant and obvious, and studies in other nearby estuaries demonstrated that erosion of marsh (and other shorelines) is a significant sediment source (though the marsh edges tend to erode more slowly, on average, than other shoreline types; Cowart et al., 2010; 2011; Currin et al, 2015).
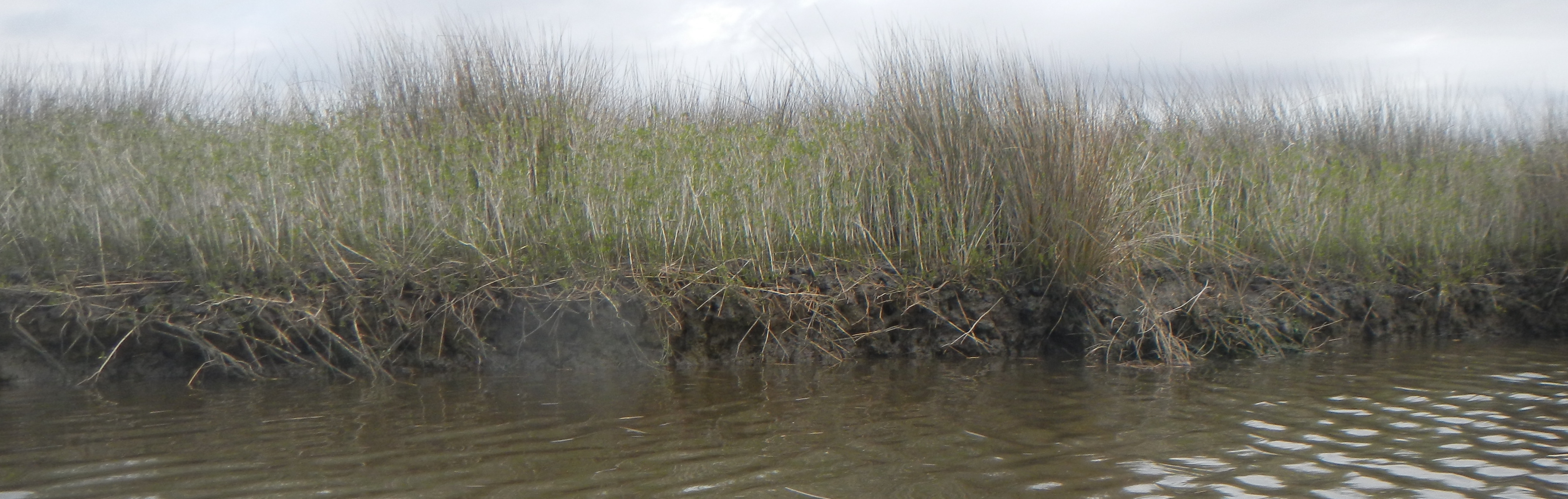
Scarped marsh bank, with high tide line clearly shown several cm above marsh surface.
6. Resuspension of bottom sediments. Like many of the tidal creeks in the region, bottom sediments are a soft, low-density mixture of mud and organics often locally referred to as “gorp.” This material is easily resuspended by waves, currents, boats, and fauna—the bottom-hugging path of turtles or alligators is often marked by a cloudy trail of resuspended sediments.
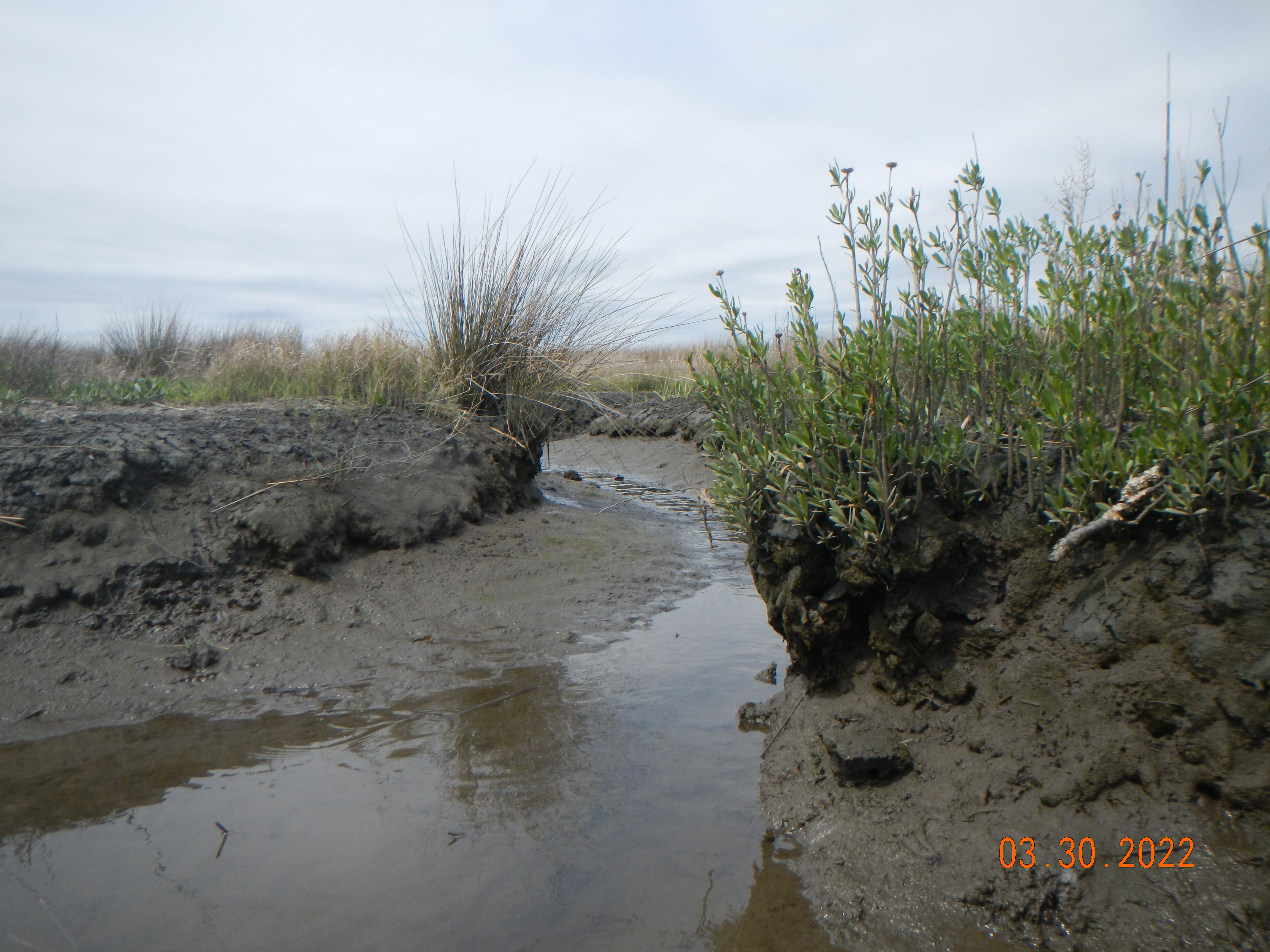
Soft bottom sediments exposed at low tide.
7. Organic matter. As in salt marshes in general, organic input from plant litter, which decomposes but slowly in the anerobic environment, is an important source—but not, of course, of mineral sediment.
Autocyclic development?
Chauhan’s (2009) paper, Autocyclic erosion in tidal marshes, resurrects (Chauhan’s term) a notion that had been overwhelmed by our fixation on changes in terrestrial sediment supplies to coastal wetlands. Essentially, Chauhan (2009) shows that marshes often undergo cycles whereby fringe erosion releases sediment, which may then be redeposited elsewhere in the marsh. At any given time, a wetland will exhibit scarped edges of recent erosion, and emergent edges where marsh is being rebuilt by progradation. Though Chauhan’s field sites were in Europe, the North River marshes are consistent with this model.
In the North River system, at low tide the maximum amount of resuspension of the benthic gorp occurs. In the shallow water, waves, turbulence, boat wakes, kayak paddles, and faunal activity stir up the sediments even more readily than at higher water levels. Much of the sediment is clay and organic matter with very low settling velocities, such that it remains suspended on the incoming tide and is delivered to the marsh surface at high tide. Even where the tidal inundation water does not become ponded so that sediment can settle out from suspension, the falling water when the ebb tide begins deposits a mud drape on the surface.
Regardless of where the sediment originally comes from, the mechanisms above are how most of it gets to the marsh surface.
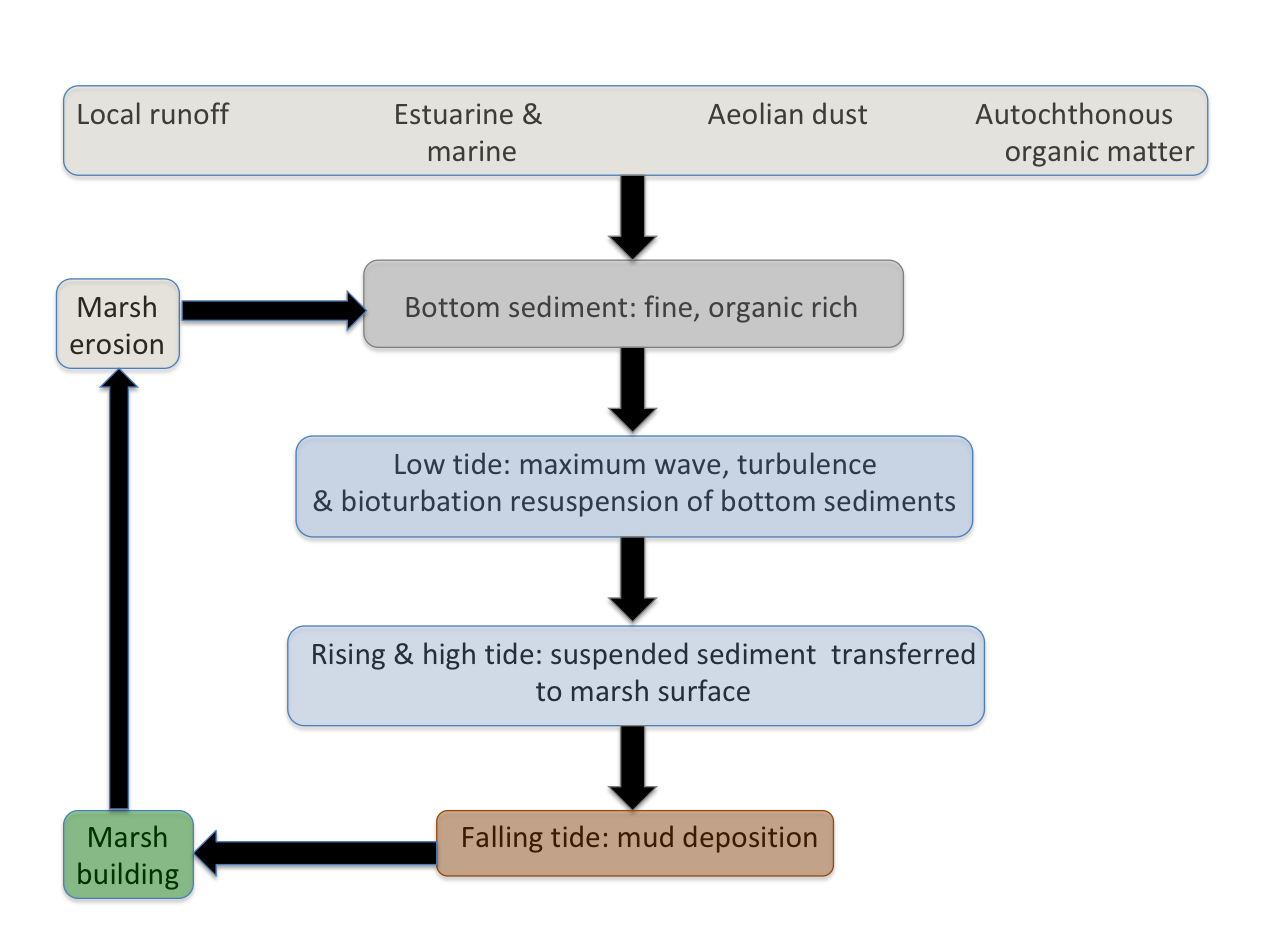
North River marsh sediment cycle.
In the discussion above, sources 1-4 are external, while 5-7 are internal. With respect to mineral sediment, bank erosion along the marshes and resuspension of bottom sediments are recycling or internally transferring sediment already delivered to the estuary/wetland system. Inland sources are irrelevant, and downstream-to-upstream transport of estuarine and marine sediment, some local inputs, and aeolian inputs all occur. The question is, how much, in both absolute and relative terms?
Sediment and sea-level
To keep pace with coastal submergence (eustatic sea-level rise plus any subsidence that may exist), wetlands must experience net surface accretion greater than or equal to the rate of coastal submergence. The extraordinarily high ecosystem services of coastal wetlands and their possible—and in many cases, ongoing—loss as sea-level rises are of clear and legitimate concern. We may be a bit too fixated on river contributions, however.
For one thing, the notion that sediment trapping behind dams—which is definitely a thing—is robbing the coastal zone of its sediment supply is overstated in general and simply untrue in some cases. But even if/where/when that is the case, it could be argued that the dam diminution of downstream sediment loads is simply returning the sediment transport regime closer to what it was before widespread human-accelerated soil erosion. A similar argument applies to marshes that grew in response to 17th and 18th century land clearing in the U.S.—their reduction may be viewed as returning the regime to its pre-disturbance state (Kirwan et al., 2015). For another thing, many salt marshes are associated with non-fluvial settings or with small coastal watersheds with limited upland or inland drainage areas.
Another complication is that the relationship between tides and sediment deposition can be quite complex. Ensign and others (2015), for example, found that the head of tide can be a bottleneck for fluvial sediment, be it sourced from near or far. If their results (from Maryland) apply to the North River, they point to estuarine sediment sources. Gunnell et al. (2013) report contemporary marsh building in the lower Newport River, where local estuarine and marine sediments are the only plausible source.
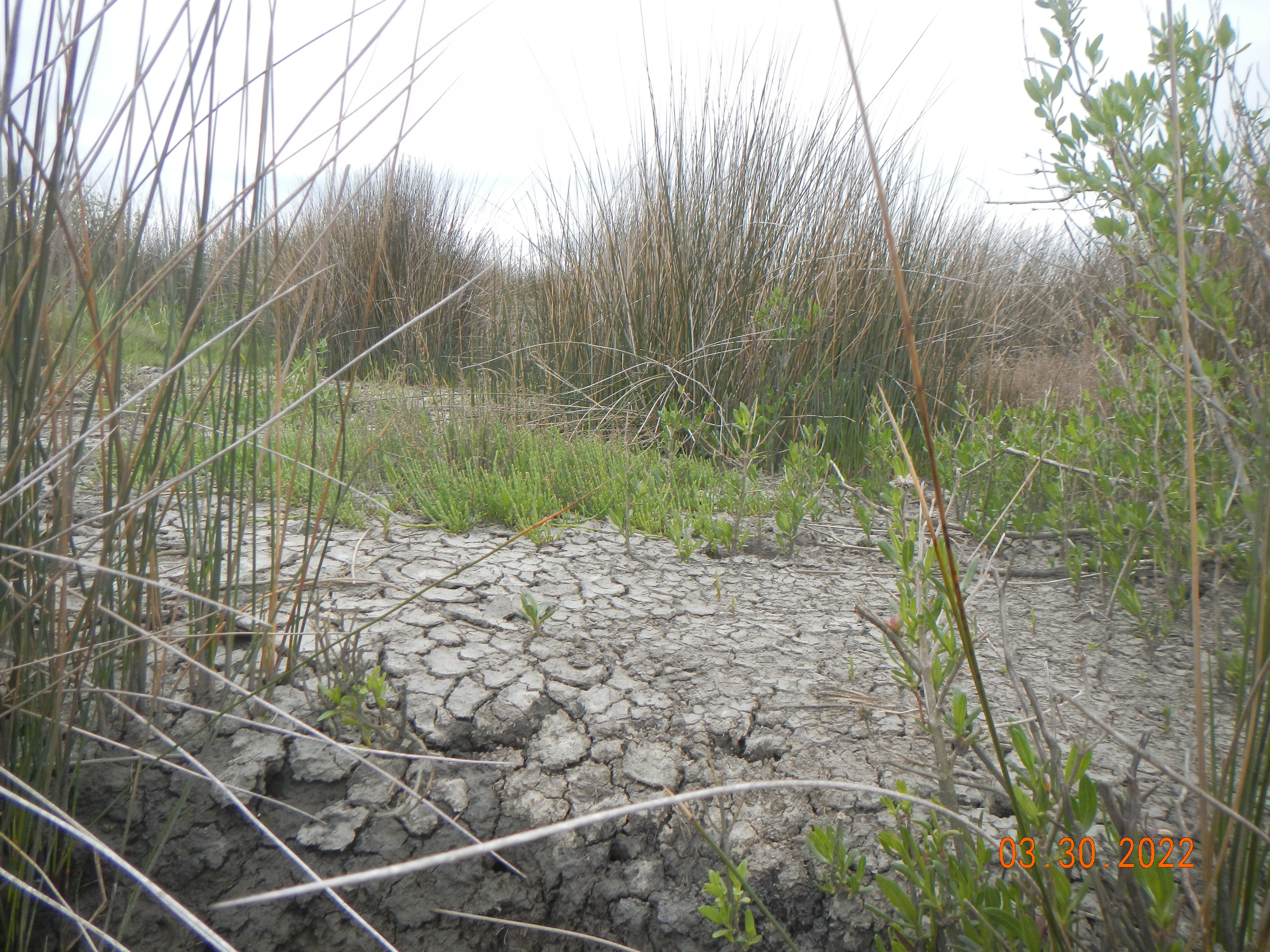
Salt pan, with saltwort (Salicornia Virginia) emerging.
It seems we have more work to do. Upland, inland sediment sources may or may not be important for salt marsh accretion, and in some cases are clearly not. And marsh erosion can occur due to internal feedbacks in the absence of coastal submergence (Chauhan, 2009; Mariotti and Fagherazzi, 2013), though in some cases an accretionary deficit is indeed the culprit.
REFERENCES
Chauhan, P.P.S. 2009. Autocyclic erosion in tidal marshes. Geomorphology 110, 45-57.
Cowart, L.; Corbett, D.R., and Walsh J.P., 2011. Shoreline change along sheltered coastlines: Insights from the Neuse River Estuary, NC, USA. Remote Sensing 3(7), 1516–1534.
Cowart, L.; Walsh, J.P., and Corbett, D.R., 2010. Analyzing estuarine shoreline change: A case study of cedar island, North Carolina. Journal of Coastal Research 26(5), 817–830.
Currin, C.A., Davis, J., Baron, L.C., et al. 2015. Shoreline change in the New River estuary, North Carolina: Rates and consequences. J. Coastal Research 31, 1069-1077.
Darwin C. 1846. An account of the fine dust which often falls on vessels in the Atlantic Ocean. The Quarterly Journal of the Geological Society of London 2 (5, Part I), 26-30.
Ensign, S.H., Noe, G.B., Hupp, C.R., Skalak, K.J. 2015. Head-of-tide bottleneck of particulate material transport from watersheds to estuaries. Geophysical Research Letters 42, 10,671–10,679, doi:10.1002/ 2015GL066830.
Gares, P.A., Slattery, M.C., Pease, P., Phillips, J.D. 2006. Eolian sediment transport on North Carolina Coastal Plain Agricultural Fields. Soil Science 171: 784-799.
Gunnell, J.R., Rodriguez, A.B., McKee, B.A. 2013. How a marsh is built from the bottom up. Geology 41, 859-862, doi:10.1130/G34582.1
Kirwan, M.L., Murray, A.B., Donnelly, J.P., Corbett, D.R. 2011. Rapid wetland expansion during European settlement and its implication for marsh survival under modern sediment delivery rates. Geology 39, 507-510.
Mariotti, G., Fagherazzi, S. 2013. Critical width of tidal flats triggers marsh collapse in the absence of sea-level rise. PNAS 11o, 6353-5356.
Mattheus, C.R., Rodriguez, A.B., McKee, B.A., 2009. Direct connectivity between upstream and downstream promotes rapid response of lower coastal-plain rivers to land-use change. Geophysical Research Letters 36, L20401. doi:10.1029/2009GL039995.
Mattheus, C.R., Rodriguez, A.B., McKee, B.A., Currin, C.A. 2010. Impact of land use change and hard structures on the evolution of fringing marsh shorelines. Estuarine, Coastal and Shelf Science 88, 365-376.
Pease, P., Gares, P.A., Lecce, S.A. 2002. Eolian dust erosion from an agricultural field on the North Carolina coastal plain. Physical Geography 23, 381-400.
Phillips, J.D., Slattery, M.C. 2006. Sediment storage, sea level, and sediment delivery to the ocean by coastal plain rivers. Progress in Physical Geography 30: 513-530.
Rodriguez, A.B., McKee, B.A., et al. 2020. Coastal sedimentation across North America doubled in the 20th century despite river dams. Nature Communications 11, 3249; doi.org/10.1038/s41467-020-16994-z |
Slattery, M.C., Phillips, J.D. 2010. Controls on sediment delivery in coastal plain rivers. Journal of Environmental Management 92: 284-289.